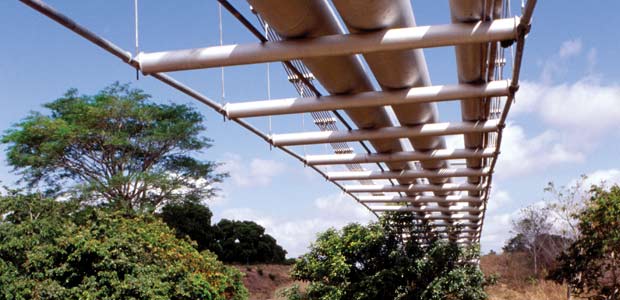
Marcellus Shale Play’s Vast Resource Potential Creating Stir In Appalachia
By Terry Engelder and Gary G. Lash
UNIVERSITY PARK, PA.–The shale gas rush is on. Excitement over natural gas production from a number of Devonian-Mississippian black shales such as the Barnett, Fayetteville and Woodford has reached the Appalachian Basin, where Range Resources has announced cumulative initial flow rates of 22 million cubic feet a day from seven horizontal wells in the Marcellus black shale play in Washington County, Pa. In fact, more than one company has announced or indicated flow rates in excess of 1 MMcf/d from vertical wells producing from the Marcellus Shale at other locations in Pennsylvania.
These reports follow on the slow, but steady success of Equitable Resources in the Big Sandy Field of Kentucky, where 20 percent of the company’s horizontal wells in the Upper Huron black shale flow without stimulation. A review of well permits reveals that horizontal laterals in both Devonian black shale plays are directed along a line striking between northwest and north-northwest. Economic flow without further stimulation is a clear indication that horizontal laterals are crossing fractures with significant connectivity to black shale matrix.
It is likely that these fractures are east-northeast striking fractures that were observed in core recovered by the Eastern Gas Shales Project (EGSP), a U.S. Department of Energy-sponsored investigation of gas potential in black shale in the Appalachian Basin. Figure 1 shows rose diagrams of the orientation of joints in ESGP core collected from the Devonian Dunkirk/Huron black shale of the Appalachian Basin. The east-northeast direction is indicated by red arrows, and the location of the Big Sandy Field is shown in the insert.
Economic gas production from black shale often requires stimulation by hydraulic fracturing with the orientation of fracture propagation controlled by the present-day earth stress. In a remarkable geological coincidence, the present-day earth stress is, to a first approximation, parallel to 300 million year-old natural fractures that allow some Huron wells of the Big Sandy Field to flow at economic rates without further stimulation. How did this economically beneficial geological coincidence come to pass in the first place? This question is best addressed by linking plate tectonics, earth stress, and the nature of fracture generation during the burial history of the Marcellus Shale.
Source Of Stress
The primary source of stress in planets with solid outer shells is the force of gravity, which pulls the planetary body inward toward a central point. Gravity acts normal to the earth’s surface, generating the vertical principal stress that is compressive within the earth. Ordinarily, a free-standing column of rock would shorten vertically under gravity, with a concomitant lateral expansion known as the Poisson effect. However, because there is no room for lateral expansion within the earth, a horizontal principal stress is generated in lieu of lateral expansion, again a consequence of the Poisson effect. This horizontal stress is less than the vertical stress because the lateral expansion is two-dimensional whereas, vertical shortening is one-dimensional. Like its vertical counterpart, horizontal stress is compressive, and increases with increasing depth in proportion to vertical stress.
A gravity-induced horizontal stress is not the only source for horizontal stress, otherwise horizontal stress would be equal in all horizontal directions; as is the case within the interior of the moon. Unlike the moon, however, the earth has an additional component of horizontal stress because the earth’s outer shell is subdivided into more than 20 large and small lithospheric plates that move laterally relative to one another. The moon’s outer shell consists of one fixed plate. The earth’s lithospheric plates move relative to the other, rubbing and grinding against one another in such a way that additional stress is transmitted to the central portion of each lithospheric plate from its boundary. This stress can add to or subtract from the gravitationally induced horizontal compressive stresses such that the magnitudes of horizontal stresses can vary in different directions. There is a maximum and minimum horizontal stress, each a principal stress, with vertical stress being another principal stress. Outside the influence of impact craters and other topography, the two horizontal stresses within the moon are theoretically equal at all times.
The generation of a component of horizontal stress beyond gravity-induced stress is inferred from the orientation of the present earth stress field. Models of stress generation based on plate shape and motion match the large intracontinental stress fields of North America, Europe and South America. Often the common denominator is plate motion, where the intracontinental maximum horizontal stress is parallel to relative motion between adjacent plates. For example, the contemporary stress in eastern North America is east-northeast and aligned with the direction of spreading between the North American and Eurasian plates.
One of the most substantial plate boundaries during the past 500 million years of Earth history is that between the present continents of Africa and North America. Once these continents were part of larger lithospheric plates called Gondwana and Laurentia, respectively. Gondwana and Laurentia were separated by a large, but rapidly closing ocean 380 million years ago. Figure 2 shows the configuration of Laurentia and Gondwana at the time of deposition of the Devonian Marcellus Shale on the continental crust of the Appalachian Basin. Note the earth’s equator indicates that Laurentia is in the southern hemisphere and rotated clockwise from its present position on the globe.
About 315 million year ago, Gondwana and Laurentia collided obliquely, and then slid past each other like hockey pucks for at least 15 million years. This glancing blow set up the stress field in the lithosphere of Laurentia that would control the orientation of fractures that formed in the Marcellus Shale and other rocks all along the 1,500-kilometer length of the Devonian-Mississippian Appalachian Basin.
Figure 3 shows the configuration of Laurentia and Gondwana during the early Alleghanian Orogeny, when the two continents were slipping past each other in a dextral sense. The orientation of dextral strike-slip faults is presented by the dashed blue line. The orientation of the lithospheric stress field at this time is shown by white arrows along with the strike of J1 joints that are controlled by this stress field.
Fracturing And Faulting
Rocks at depths where hydrocarbons form may respond to earth stress by some combination of brittle fracture and ductile flow. The former process encompasses two styles of rupture, depending on the motion of the walls of the fracture. Faults are the product of a sliding or tearing motion parallel to the walls of the fracture. This style of rupture may be so fast that seismic noise is released in the form of an earthquake. Joints, the product of the other rupture style, are the result of splitting where motion of the walls of the fracture is normal to the plane of the rupture.
Figure 4 shows an example of two cross-cutting joint sets in the Geneseo black shale of the Appalachian Basin (this outcrop is in a bed of the Taughannock Creek, north of Ithaca, N.Y.). Splitting is often a slow process, releasing little to no seismic noise. Joints and faults form in distinct and predictable orientations relative to the responsible earth stress.
In addition to the differences between the Earth and the moon, the character of planetary stress is the product of a solid outer shell and is, for example, much different from the “stress” found in the liquid outer shell of Jupiter. Rock, like all solids, can maintain its shape against the force of gravity, whereas fluids are not free standing, as the turbulent outer shell of Jupiter or the Earth’s oceans testify. For solids, the ability to resist the force of gravity means that internal stress can vary with orientation whereas pressure, the term for stress in a liquid, is equal in all directions in a fluid. If internal stress varies with orientation, the solid is subject to shear stress.
However, in a solid, there are three mutually perpendicular planes along which shear stress does not act. All other planes in a solid are subject to a shear stress, which may become large enough to cause rupture-parallel slip (i.e., a fault). A rupture that opens without slip (i.e., a joint) propagates along one of those shear stress-free mutually perpendicular planes, and that one plane is normal to the least compressive stress, a principal stress.
A net tension is required for joint propagation, a rare condition at depth in an earth pulled inward by the force of gravity. Joints at depth in the earth are driven by a pore pressure that exceeds the least compressive stress, a mechanism called natural hydraulic fracture. Regardless of whether joints form in true tension near the earth’s surface or by natural hydraulic fracturing within the oil window, the orientation of their plane is a fool-proof indicator of the orientation of earth stress at the time of propagation. A vertical joint propagates parallel to the maximum horizontal compressive stress at the time of propagation and its normal points in the direction of least compressive stress.
Burial History
The Marcellus Shale accumulated on continental crust in a relatively shallow (less than 200 meters) interior seaway, perhaps because sea level was unusually high at this time, as indicated in Figure 2. At the time of deposition of the Marcellus Shale about 380 million years ago, Gondwana was rushing toward Laurentia at such a rate that thrust faulting caused crustal thickening in a highland at the edge of the continent. The drawing in Figure 5 shows the sinking of the Appalachian Basin seabed as a consequence of thrust loading during the convergence of Gondwana toward Laurentia. Thickening at the edge of Laurentia constituted a load that bent the continental margin much like the weight of a diver bends a diving board.
During crustal loading, the seabed of the Appalachian Basin sank below a pycnocline, a boundary in the ocean that separates warm, oxygenated surface water from cooler, oxygen-deficient water deeper in the ocean. An episode of thrust loading disrupted river systems so that, for a period, sediment flux into the basin was low, favoring the accumulation of rock with a high total organic carbon (TOC) content. Eventually, river channels organized to deliver clastic sediments at a higher rate so the gray shale covered the black shale. This cycle of thrust loading and concomitant black shale deposition repeated at least eight times during a period of 20 million years. Figure 6 shows the eight Devonian black shales found in the Appalachian Basin.
During much of the period leading up to the collision of Gondwana and Laurentia, sedimentation rate was high, occasionally in excess of 150 meters per million years. Ordinarily, seawater is squeezed out of pore space during burial, but a high sedimentation rate does not allow time for pore water to escape the fine-grained matrix of the black and gray shale. Because water is incompressible relative to the shale matrix, this trapped seawater supports the weight of additional sedimentation, preventing further compaction of pore space. Pore pressure must increase when supporting the weight of sedimentation. This process, called compaction disequilibrium, is the first mechanism to generate abnormally high fluid pressure in the Devonian section, including the Marcellus Shale.
Burial of the Marcellus continued with a concomitant increase in temperature and pressure until the oil window was reached approximately 300 million years ago. Oil and gas were generated from organic matter by a chemical reaction that ordinarily requires an increase in pore space. However, because pore space did not expand during burial of the Marcellus Shale, the generation of oil and gas in this organic-rich unit resulted in an additional increment of pore pressure. Progressive production of oil and gas in the Marcellus increased pore to such a magnitude that the pressure was relieved by expansion of the rock through cracking, starting with microcracks around flakes of organic matter.
Figure 7 shows cracks propagating from kerogen flakes in Devonian black shale. These cracks are driven by pressure developed around the kerogen flakes by the chemical reaction that converts kerogen to gas and oil. As more hydrocarbon is generated, the cracks continue to grow until they open into full scale joints (i.e., J1) that are natural hydraulic fractures. Figure 8 is a natural hydraulic fracture driven by gas with the compressibility of methane–in this case, a J2 joint in the Ithaca formation of the Genesee Group exposed at Watkins Glen, N.Y. The rupture propagated from right to left, as indicated by plumose morphology showing two increments with surface roughness increasing until arrest.
J1 Joints
Initial hydraulic fracturing was restricted to the Marcellus and other black shale source rocks of the Appalachian Basin. Early cracking was in the plane of bedding, largely because the microscopic strength anisotropy generated by early compaction favored horizontal microcracks. Fluid within this suite of microcracks eventually collected to drive mesoscopic scale joints that were separated by as little as 30-50 centimeters. At this point, the orientation of these joints was controlled by the earth’s stress field, principally the least compressive stress.
During initial hydrocarbon generation, Gondwana was slipping obliquely past Laurentia, thereby setting up a continental-scale stress field in the Appalachian Basin. At this time, the maximum horizontal stress was west/northwest to west/northwest-east/southeast and at a small acute angle to the strike-slip faults that distributed slip between Gondwana and Laurentia. This stress field not only controlled joints in the black shale, but also the orientation of early face cleat in younger coals in the Appalachian Basin. It is this stress field that controlled the orientation of the joint set that allows Equitable Resources to produce gas from some of its horizontal wells in the Big Sandy Field without stimulation.
By about 290 million years ago, dextral strike-slip motion of Gondwana relative to Laurentia was arrested when a continental promontory in the vicinity of New York City locked Gondwana and Laurentia at a pivot point. Figure 9 shows the configuration of Laurentia and Gondwana after the continents had locked at the New York promontory (indicated by the large red dot). For the next 15 million years or so, Gondwana spun in a clockwise fashion around the New York promontory.
This clockwise rotation drove Gondwana into Laurentia southwest of the pivot point to create the foreland fold-thrust belts of the Central and Southern Appalachians during a mountain building event called the Alleghanian Orogeny. The pivoting of Gondwana resulted in the reorganization of the intracontinental stress field of Laurentia such that the maximum horizontal stress was nearly perpendicular to its orientation by the time J1 joints had formed.The Appalachians northeast of the pivot point display the remnants of the strike-slip tectonics, but lack the folds and faults of the Central and Southern Appalachians. In Figure 9, the orientation of the lithospheric stress field is indicated by the white arrows, along with the strike of J2 joints that are in the cross-fold orientation relative to the Appalachian Mountains.
During the period between the deposition of the Marcellus black shale and the Alleghanian Orogeny, the Appalachian Basin was in the southern hemisphere and oriented as much as 60 degrees clockwise from its present orientation. After the end of the Alleghanian Orogeny, plate tectonics carried North America with the Appalachian Basin into the northern hemisphere in a motion that spun the continent counter-clockwise to its present position.
Natural Hydraulic Fractures
During the Alleghanian Orogeny, the Marcellus was further buried, resulting in a continuation of the generation of hydrocarbons. Fluid pressure continued to build to such a level that natural hydraulic fractures were driven upward out of the Marcellus and other black shales and into the overlying gray shale succession. In some places, jointing was localized to form gas chimneys that are remarkable for their height, which extends vertically off the top of black shale units at least 50 meters. Joints (J2) in overlying gray shale propagated in the stress field set up by the collision of Gondwana as it pivoted clockwise into Laurentia, cutting the older J1 joints in the black shales at a high angle to J1.
An example of J2 joints cutting up-section from a black shale (i.e., the Devonian Geneseo formation at Taughannock Falls State Park in New York into gray shales of the Ithaca formation of the Genesee Group) is shown in Figure 10. The height-to-spacing ratio of these joints is indicative of natural hydraulic fracturing.
Several lines of evidence point to the propagation of both J1 and J2 as natural hydraulic fractures. First, tensile joints cleave carbonate concretions whereas the concretion acts as a barrier for natural hydraulic fractures. A natural hydraulic fracture will propagate around the concretion while leaving the concretion intact. Figure 11 is an example of a J1 joint cutting a black shale (i.e, the Devonian Rhinestreet along Eighteenmile Creek in Erie County, N.Y.) without cleaving carbonate concretions. This is one of the characteristic behaviors of a natural hydraulic fracture.
Second, tensile stress will cause continuous crack propagation, whereas internal pressure will lead to incremental propagation and arrest. The rupture process in rocks is irregular on a microscopic scale, while the overall surface of a joint remains planar. An irregular rupture prints a surface morphology or plumose structure on the surface of joints. The depth of the irregularity varies with velocity of the rupture, so that when a joint propagates in occasional spurts, there is an unmistakable characteristic pattern of starts and stops.
Episodic joint propagation is best understood using Boyles Law for the behavior of an ideal gas: P1V1 = P2V2, where P is pressure and V is volume. If a joint ruptures in a spurt, the volume suddenly goes up, and by Boyles Law, the pressure inside the joint would decrease. Incremental rupture indicates that pressure builds again until the rupture starts anew. However, during each increment of propagation, the source of fluid cannot feed fluid to the growing joint at a rate that keeps up with joint propagation. The mechanism by which fluid is fed to the joint volume is through the pore space on either side of the joint. The decrease of pressure within the joint after each cycle leads to an inward pressure that drives flow from the rock matrix to the open joint. Fluid filling the joint causes pressure to rise until rupture commences again, cycle after cycle. As many as 68 cycles have been counted on one joint interface.
Another characteristic of propagation within the shale sequence of the Catskill Delta is that rupture increment length gradually increases with length of the joint. Increment length scales with the thickness of the bed with initial lengths shorter than bed thickness. Through several dozen cycles, the increment length exceeds bed thickness. This behavior is characteristic of a compressible gas such as methane. In fact, it comes as no surprise to find that methane drove natural hydraulic fracturing in the Catskill Delta complex, given the long history of hydrocarbon maturation as the Appalachian Basin was buried to depths to five kilometers. J1 and J2 are rarely filled with precipitated minerals, meaning that methane was retained during the subsequent 250 million year history of the basin.
Size Of Marcellus Resource
During the past several months, a number of estimates concerning the size of the Marcellus play have been published. Several reputable reporters have confused various measures of gas in the Marcellus Shale. Gas in-place is the total amount of free and adsorbed gas within the Marcellus. Given a resource that is found under more than 34,000,000 acres of real estate with at least 50 feet of organic-rich section, the Marcellus Shale weighs in with more than 500 trillion cubic feet of gas in-place spread over a four state area. Continuous natural gas accumulations such as the Barnett Shale produce more than 10 percent of the gas in-place, which when applied to the Marcellus Shale, translates to a resource that will return 50 Tcf in time.
Confusion arises when this figure for technically recoverable gas is compared with the U.S. Geological Survey’s prediction of 1.9 Tcf for an undiscovered resource in a portion of the Marcellus. The two numbers should not be compared, since the USGS figure relies heavily on knowledge of the ultimate recoverable gas per well. Because there has been little production from the Marcellus, the USGS figure is inherently low, but will begin to climb when production comes on line.
Production from the Huron/Dunkirk interval of the Big Sandy Field has enabled the USGS to predict an undiscovered resource of 6.3 Tcf. This field has less than 25 percent of acreage found within the boundaries of the Marcellus play (Figure 12), and the average depth of the Big Sandy Field is less than that of the heart of the Marcellus play. The scaling factor between the Big Sandy Field and the Marcellus play is about eight, which means that all else being equal, extrapolating the Dunkirk/Huron play suggests a total resource of the Marcellus play of nearly 50 Tcf. With this extrapolation, the USGS and Engelder-Lash estimates are in agreement.
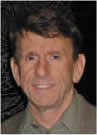
TERRY ENGELDER is a professor of geosciences in the Department of Geosciences at Pennsylvania State University in University Park, Pa. Before joining the university in 1985, Engelder served at Columbia University’s Lamont-Doherty Earth Observatory, the United States Geological Survey, and Texaco. He holds a B.S. in geology from Pennsylvania State University, an M.S. in geology from Yale University, and a Ph.D. in geology from Texas A&M University.
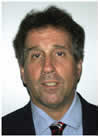
GARY G. LASH is a professor in the Department of Geosciences at the State University of New York at Fredonia. Before joining the university in 1981, Lash served at the Virginia Division of Mineral Resources and the United States Geological Survey. He holds a B.A. in geology from Kutztown State University, and an M.S. and Ph.D. in geology from Lehigh University.
For other great articles about exploration, drilling, completions and production, subscribe to The American Oil & Gas Reporter and bookmark www.aogr.com.